A new electrode design boosts the efficiency of electrochemical reactions that turn carbon dioxide into ethylene and other products.
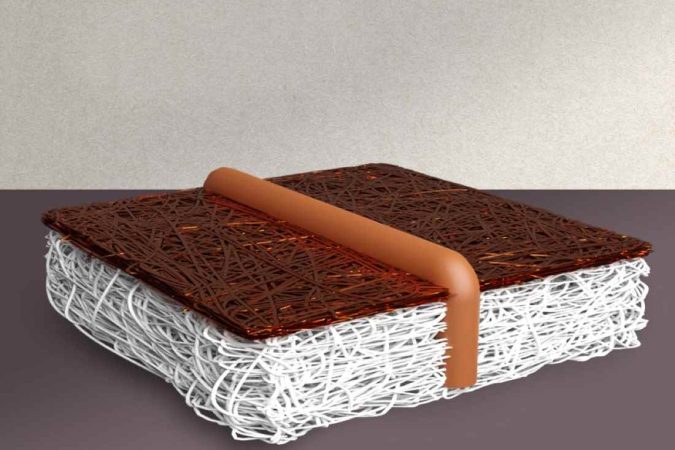
CAMBRIDGE, Mass. — As the world struggles to reduce greenhouse gas emissions, researchers are seeking practical, economical ways to capture carbon dioxide and convert it into useful products, such as transportation fuels, chemical feedstocks, or even building materials. But so far, such attempts have struggled to reach economic viability.
New research by engineers at MIT could lead to rapid improvements in a variety of electrochemical systems that are under development to convert carbon dioxide into a valuable commodity. The team developed a new design for the electrodes used in these systems, which increases the efficiency of the conversion process.
The findings will be reported in the journal Nature Communications, in a paper by MIT doctoral student Simon Rufer, professor of mechanical engineering Kripa Varanasi, and three others.
“The CO2 problem is a big challenge for our times, and we are using all kinds of levers to solve and address this problem,” Varanasi says. It will be essential to find practical ways of removing the gas, he says, either from sources such as power plant emissions, or straight out of the air or the oceans. But then, once the CO2 has been removed, it has to go somewhere.
A wide variety of systems have been developed for converting that captured gas into a useful chemical product, Varanasi says. “It’s not that we can’t do it — we can do it. But the question is how can we make this efficient? How can we make this cost-effective?”
In the new study, the team focused on the electrochemical conversion of CO2 to ethylene, a widely used chemical that can be made into a variety of plastics as well as fuels, and which today is made from petroleum. But the approach they developed could also be applied to producing other high-value chemical products as well, including methane, methanol, carbon monoxide, and others, the researchers say.
Currently, ethylene sells for about $1,000 per ton, so the goal is to be able to meet or beat that price. The electrochemical process that converts CO2 into ethylene involves a water-based solution and a catalyst material, which come into contact along with an electric current in a device called a gas diffusion electrode.
There are two competing characteristics of the gas diffusion electrode materials that affect their performance: They must be good electrical conductors so that the current that drives the process doesn’t get wasted through resistance heating, but they must also be “hydrophobic,” or water repelling, so the water-based electrolyte solution doesn’t leak through and interfere with the reactions taking place at the electrode surface.
Unfortunately, it’s a tradeoff. Improving the conductivity reduces the hydrophobicity, and vice versa. Varanasi and his team set out to see if they could find a way around that conflict, and after many months of trying, they did just that.
The solution, devised by Rufer and Varanasi, is elegant in its simplicity. They used a plastic material, PTFE (essentially Teflon), that has been known to have good hydrophobic properties. However, PTFE’s lack of conductivity means that electrons must travel through a very thin catalyst layer, leading to significant voltage drop with distance. To overcome this limitation, the researchers wove a series of conductive copper wires through the very thin sheet of the PTFE.
“This work really addressed this challenge, as we can now get both conductivity and hydrophobicity,” Varanasi says.
Research on potential carbon conversion systems tends to be done on very small, lab-scale samples, typically less than 1-inch (2.5-centimeter) squares. To demonstrate the potential for scaling up, Varanasi’s team produced a sheet 10 times larger in area and demonstrated its effective performance.
To get to that point, they had to do some basic tests that had apparently never been done before, running tests under identical conditions but using electrodes of different sizes to analyze the relationship between conductivity and electrode size. They found that conductivity dropped off dramatically with size, which would mean much more energy, and thus cost, would be needed to drive the reaction.
“That’s exactly what we would expect, but it was something that nobody had really dedicatedly investigated before,” Rufer says. In addition, the larger sizes produced more unwanted chemical byproducts besides the intended ethylene.
Real-world industrial applications would require electrodes that are perhaps 100 times larger than the lab versions, so adding the conductive wires will be necessary for making such systems practical, the researchers say. They also developed a model which captures the spatial variability in voltage and product distribution on electrodes due to ohmic losses. The model along with the experimental data they collected enabled them to calculate the optimal spacing for conductive wires to counteract the drop off in conductivity.
In effect, by weaving the wire through the material, the material is divided into smaller subsections determined by the spacing of the wires. “We split it into a bunch of little subsegments, each of which is effectively a smaller electrode,” Rufer says. “And as we’ve seen, small electrodes can work really well.”
Because the copper wire is so much more conductive than the PTFE material, it acts as a kind of superhighway for electrons passing through, bridging the areas where they are confined to the substrate and face greater resistance.
To demonstrate that their system is robust, the researchers ran a test electrode for 75 hours continuously, with little change in performance. Overall, Rufer says, their system “is the first PTFE-based electrode which has gone beyond the lab scale on the order of 5 centimeters or smaller. It’s the first work that has progressed into a much larger scale and has done so without sacrificing efficiency.”
The weaving process for incorporating the wire can be easily integrated into existing manufacturing processes, even in a large-scale roll-to-roll process, he adds.
“Our approach is very powerful because it doesn’t have anything to do with the actual catalyst being used,” Rufer says. “You can sew this micrometric copper wire into any gas diffusion electrode you want, independent of catalyst morphology or chemistry. So, this approach can be used to scale anybody’s electrode.”
“Given that we will need to process gigatons of CO2 annually to combat the CO2 challenge, we really need to think about solutions that can scale,” Varanasi says. “Starting with this mindset enables us to identify critical bottlenecks and develop innovative approaches that can make a meaningful impact in solving the problem. Our hierarchically conductive electrode is a result of such thinking.”
The research team included MIT graduate students Michael Nitzsche and Sanjay Garimella, as well as Jack Lake PhD ’23. The work was supported by Shell, through the MIT Energy Initiative.